25.2 Metabolism in Context: Glycogen Breakdown and Synthesis Are Reciprocally Regulated
✓ 5 Describe how glycogen mobilization and synthesis are coordinated.
An important control mechanism prevents glycogen from being synthesized at the same time as it is being broken down. The same glucagon- and epinephrine-triggered cAMP cascades that initiate glycogen breakdown in the liver and muscle, respectively, also shut off glycogen synthesis. Glucagon and epinephrine control both glycogen breakdown and glycogen synthesis through protein kinase A (Figure 25.3). Recall that protein kinase A adds a phosphoryl group to phosphorylase kinase, activating that enzyme and initiating glycogen breakdown. Glycogen synthase kinase and protein kinase A add a phosphoryl group to glycogen synthase, but this phosphorylation leads to a decrease in enzymatic activity. In this way, glycogen breakdown and synthesis are reciprocally regulated. How is the enzymatic activity of glycogen phosphorylase reversed so that glycogen breakdown halts and glycogen synthesis begins?
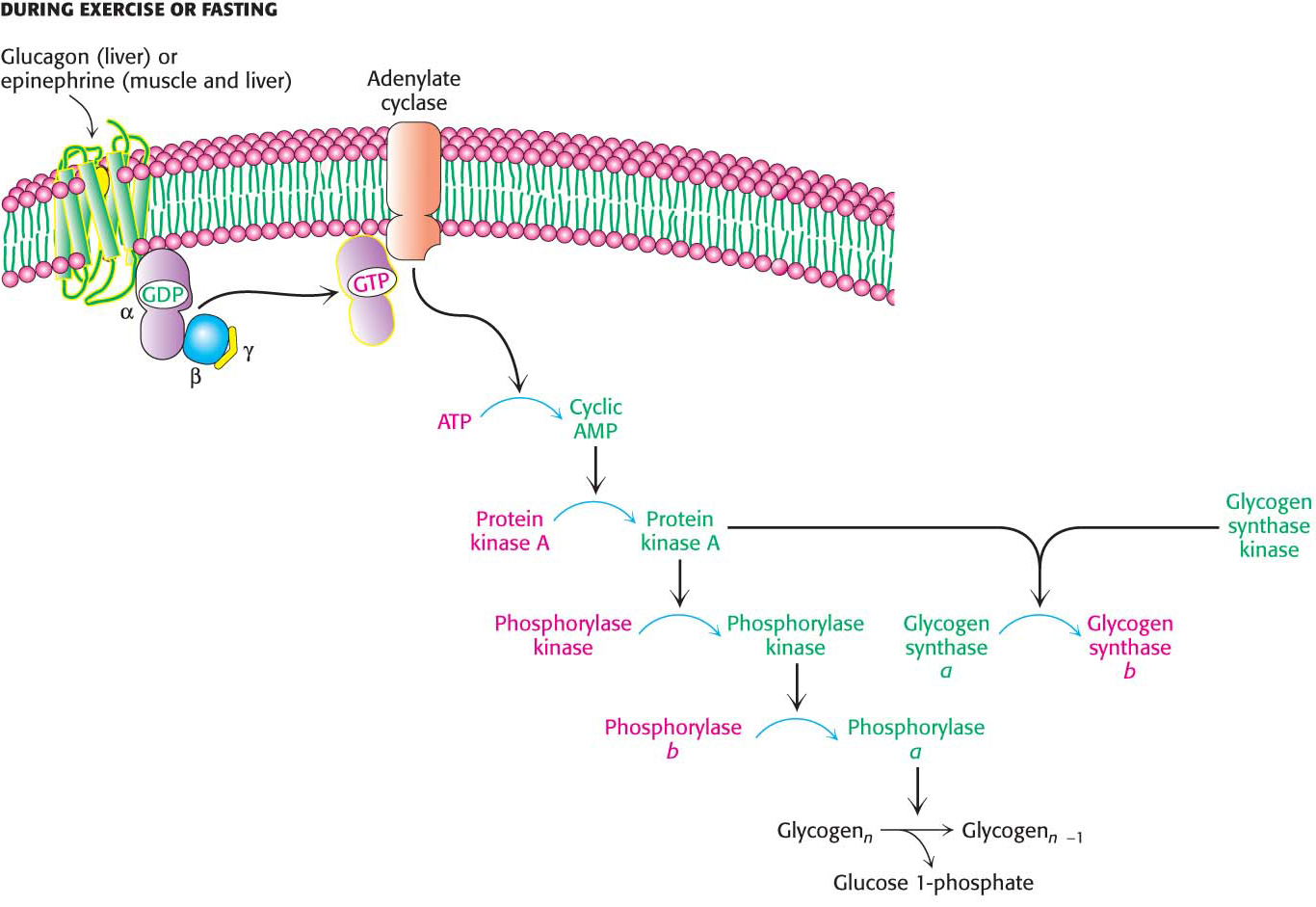
Figure 25.3: Coordinate control of glycogen metabolism. Glycogen metabolism is regulated, in part, by hormone-triggered cyclic AMP cascades. The sequence of reactions leading to the activation of protein kinase A ultimately activates glycogen degradation. At the same time, protein kinase A, along with glycogen synthase kinase, inactivates glycogen synthase, shutting down glycogen synthesis.
Protein Phosphatase 1 Reverses the Regulatory Effects of Kinases on Glycogen Metabolism
After a bout of exercise, muscle must shift from a glycogen-degrading mode to one of glycogen replenishment. A first step in this metabolic task is to shut down the phosphorylated proteins that stimulate glycogen breakdown. This step is accomplished by protein phosphatases that catalyze the hydrolysis of phosphorylated serine and threonine residues in proteins. Protein phosphatase 1 (PP1) plays key roles in regulating glycogen metabolism (Figure 25.4). PP1 inactivates phosphorylase a and phosphorylase kinase by dephosphorylating them. PP1 there by decreases the rate of glycogen breakdown; it reverses the effects of the phosphorylation cascade. Moreover, PP1 removes phosphoryl groups from glycogen synthase b to convert it into the more active glycogen synthase a form. Here, PP1 also accelerates glycogen synthesis. PP1 is yet another molecular device in the coordinate control of glycogen metabolism.
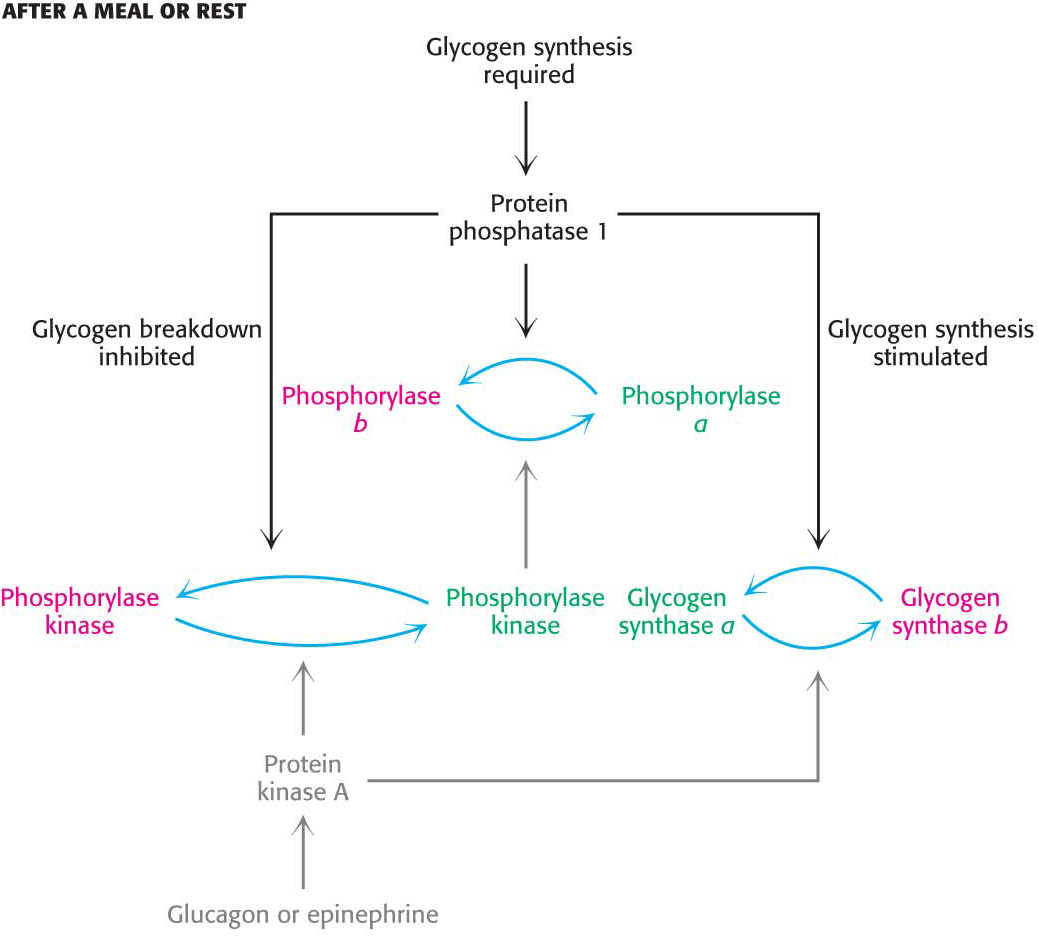
Figure 25.4: The regulation of glycogen synthesis by protein phosphatase 1. PP1 stimulates glycogen synthesis while inhibiting glycogen breakdown.
The catalytic subunit of PP1 is a single-domain protein. This subunit is usually bound to one of a family of regulatory subunits; in skeletal muscle and the heart, the most prevalent regulatory subunit is called GM, whereas, in the liver, the most prevalent subunit is GL. These regulatory subunits have multiple domains that participate in interactions with glycogen, with the catalytic subunit of the protein phosphatase, and with target enzymes. Thus, these regulatory subunits act as scaffolds, bringing together the protein phosphatase and its substrates in the context of a glycogen particle.
What prevents the phosphatase activity of PP1 from always inhibiting glycogen degradation? When glycogen degradation is called for, epinephrine or glucagon initiates the cAMP cascade that activates protein kinase A (Figure 25.5). Protein kinase A reduces the activity of PP1 by two mechanisms. First, in muscle, GM is phosphorylated, resulting in the release of the catalytic subunit. Dephosphorylation is therefore greatly reduced. Second, almost all tissues contain small proteins that, when phosphorylated, inhibit the catalytic subunit of PP1. Thus, when glycogen degradation is switched on by cAMP, the phosphorylation of these inhibitors shuts off protein phosphatases, keeping glycogen phosphorylase in its active a form and glycogen synthase in its inactive b form.
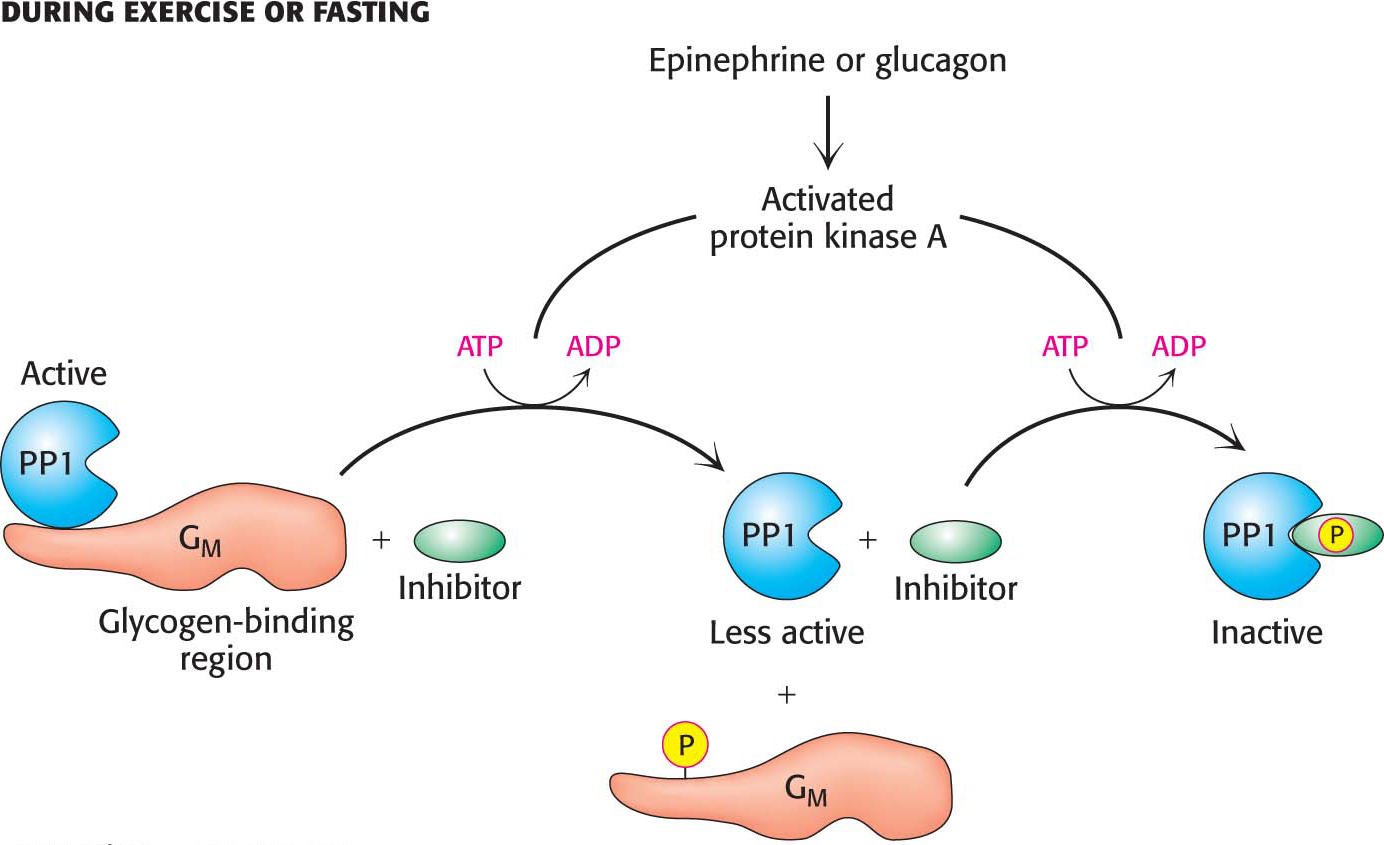
Figure 25.5: The regulation of protein phosphatase 1 in muscle takes place in two steps. Phosphorylation of GM by protein kinase A dissociates the catalytic subunit from its substrates in the glycogen particle. Phosphorylation of the inhibitor subunit by protein kinase A inactivates the catalytic unit of PP1.
Insulin Stimulates Glycogen Synthesis by Inactivating Glycogen Synthase Kinase
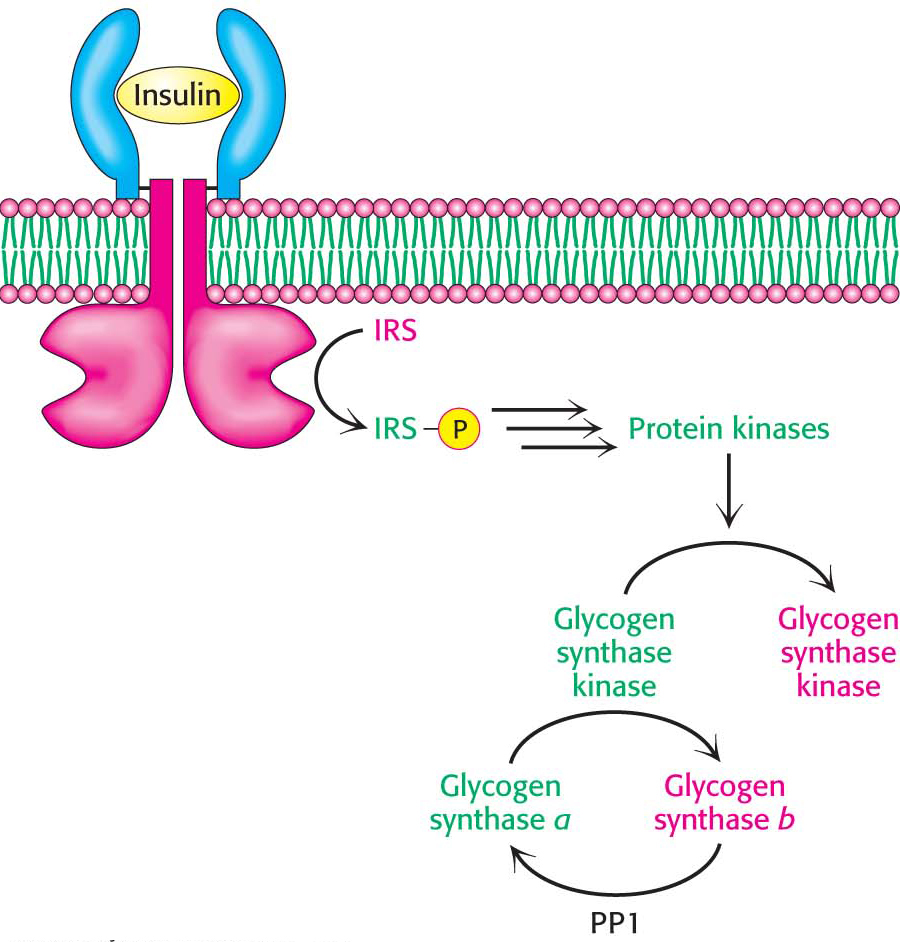
Figure 25.6: Insulin inactivates glycogen synthase kinase. Insulin triggers a cascade that leads to the phosphorylation and inactivation of glycogen synthase kinase and prevents the phosphorylation of glycogen synthase. Protein phosphatase 1 (PP1) removes the phosphoryl groups from glycogen synthase, thereby activating the enzyme and allowing glycogen synthesis. Abbreviation: IRS, insulin-receptor substrate.
After exercise, people often consume carbohydrate-rich foods to restock their glycogen stores. Indeed, the primary means of clearing glucose from the blood in human beings is its conversion into muscle glycogen. How is glycogen synthesis stimulated? When blood-glucose concentration is high, insulin stimulates the synthesis of glycogen. This stimulation has two components. First, recall that insulin leads to an increase in the amount of glucose in the cell by increasing the number of glucose transporters (GLUT4) in the cell membrane. The entry of glucose and its subsequent conversion into glucose 6-phosphate allosterically activates glycogen synthase b. Second, insulin leads to the inactivation of glycogen synthase kinase, the enzyme that maintains glycogen synthase in its phosphorylated, less active state (Figure 25.6).
How does insulin exert its effects? The first step in the action of insulin is binding to its receptor tyrosine kinase in the plasma membrane. The binding of insulin activates the tyrosine kinase activity of the receptor so that it phosphorylates insulin-receptor substrates. These phosphorylated proteins trigger signal-transduction pathways that eventually lead to the movement of glucose transporters to the cell membrane and to the activation of protein kinases that phosphorylate and inactivate glycogen synthase kinase. The inactive kinase can no longer maintain glycogen synthase in its phosphorylated, less active state. In the meantime, protein phosphatase 1 dephosphorylates glycogen synthase, further stimulating the enzyme and restoring glycogen reserves. The net effect of insulin is the replenishment of glycogen stores.
Glycogen Metabolism in the Liver Regulates the Blood-Glucose Concentration
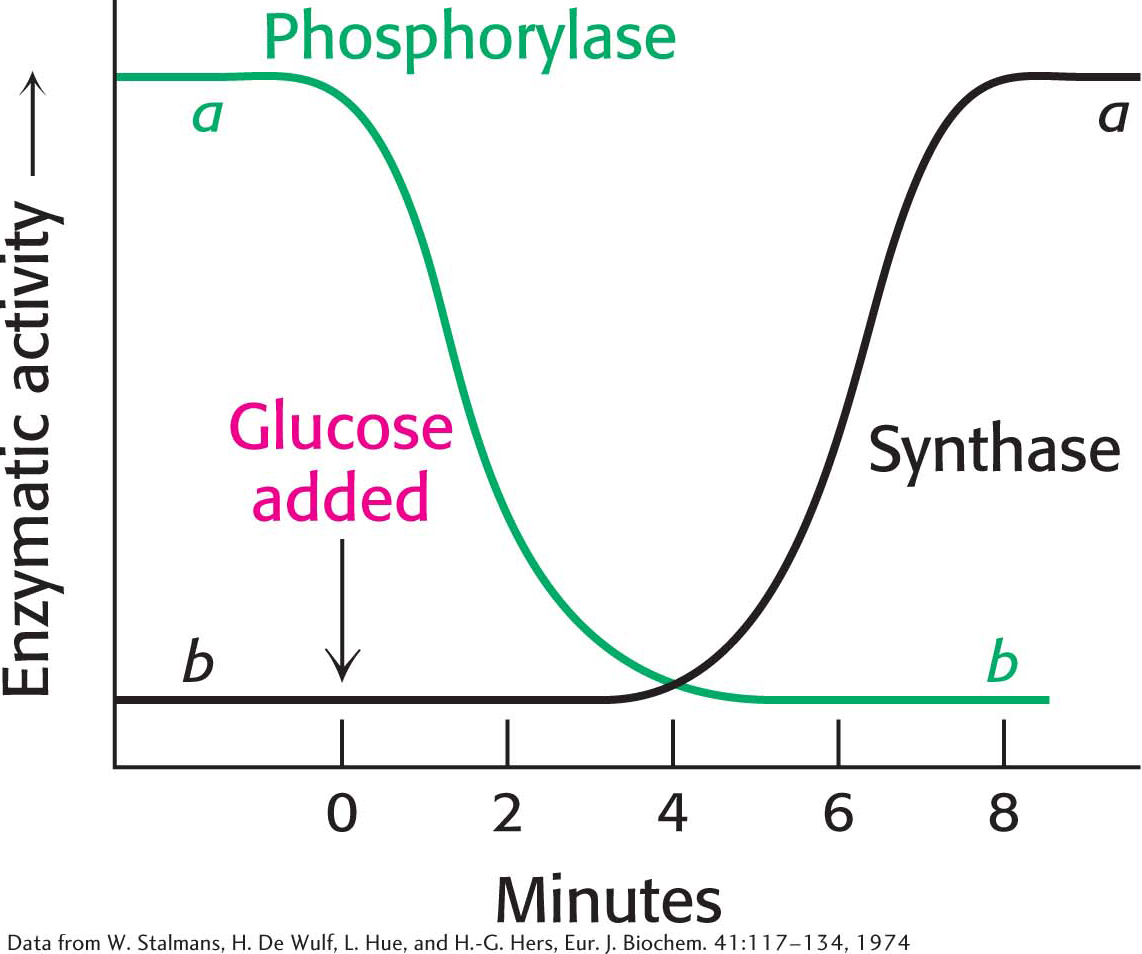
Figure 25.7: Blood glucose regulates liver-glycogen metabolism. The infusion of glucose into the bloodstream leads to the inactivation of phosphorylase followed by the activation of glycogen synthase in the liver.
After a meal rich in carbohydrates, blood-glucose concentration rises, and glycogen synthesis is increased in the liver. Although insulin is the primary signal for glycogen synthesis, another signal is the concentration of glucose in the blood, which normally ranges from 4.4 to 6.7 mM. The liver senses the concentration of glucose in the blood and takes up or releases glucose to maintain a normal concentration. The amount of liver phosphorylase a decreases rapidly when glucose is infused into the blood (Figure 25.7). In fact, phosphorylase a is the glucose sensor in liver cells. The binding of glucose to phosphorylase a shifts its allosteric equilibrium from the active R form to the inactive T form. This conformational change renders the phosphoryl group on serine 14 a substrate for protein phosphatase 1. PP1 binds tightly to phosphorylase a only when the phosphorylase is in the R state, but PP1 is inactive when bound. When glucose induces the transition to the T form, PP1 dissociates from the phosphorylase and becomes active, converting liver phosphorylase a into the b form. Recall that the R⇌T transition of muscle phosphorylase a is unaffected by glucose and is thus unaffected by the rise in blood-glucose concentration.
After a period in which the amount of glycogen phosphorylase a is decreasing, the amount of glycogen synthase a increases, resulting in glycogen being synthesized from the glucose that has now entered the liver. How does glucose activate glycogen synthase? Phosphorylase b, in contrast with phosphorylase a, does not bind the phosphatase. Consequently, the conversion of a into b is accompanied by the release of PP1, which is then free to activate glycogen synthase and inactivate glycogen phosphorylase (Figure 25.8). The removal of the phosphoryl group of inactive glycogen synthase b converts it into the active a form. Initially, there are about 10 phosphorylase a molecules per molecule of phosphatase. Consequently, most of the phosphorylase a is converted into b before any phosphatase is released. Hence, the activity of glycogen synthase begins to increase only after most of the phosphorylase is inactivated (Figure 25.7). The lag between degradation and synthesis prevents the two pathways from operating simultaneously. This remarkable glucose-sensing system depends on three key elements: (1) communication between the allosteric site for glucose and the serine phosphate, (2) the use of PP1 to inactivate phosphorylase and activate glycogen synthase, and (3) the binding of the phosphatase to phosphorylase a to prevent the premature activation of glycogen synthase.
!quickquiz! QUICK QUIZ 2
What accounts for the fact that liver phosphorylase is a glucose sensor, whereas muscle phosphorylase is not?
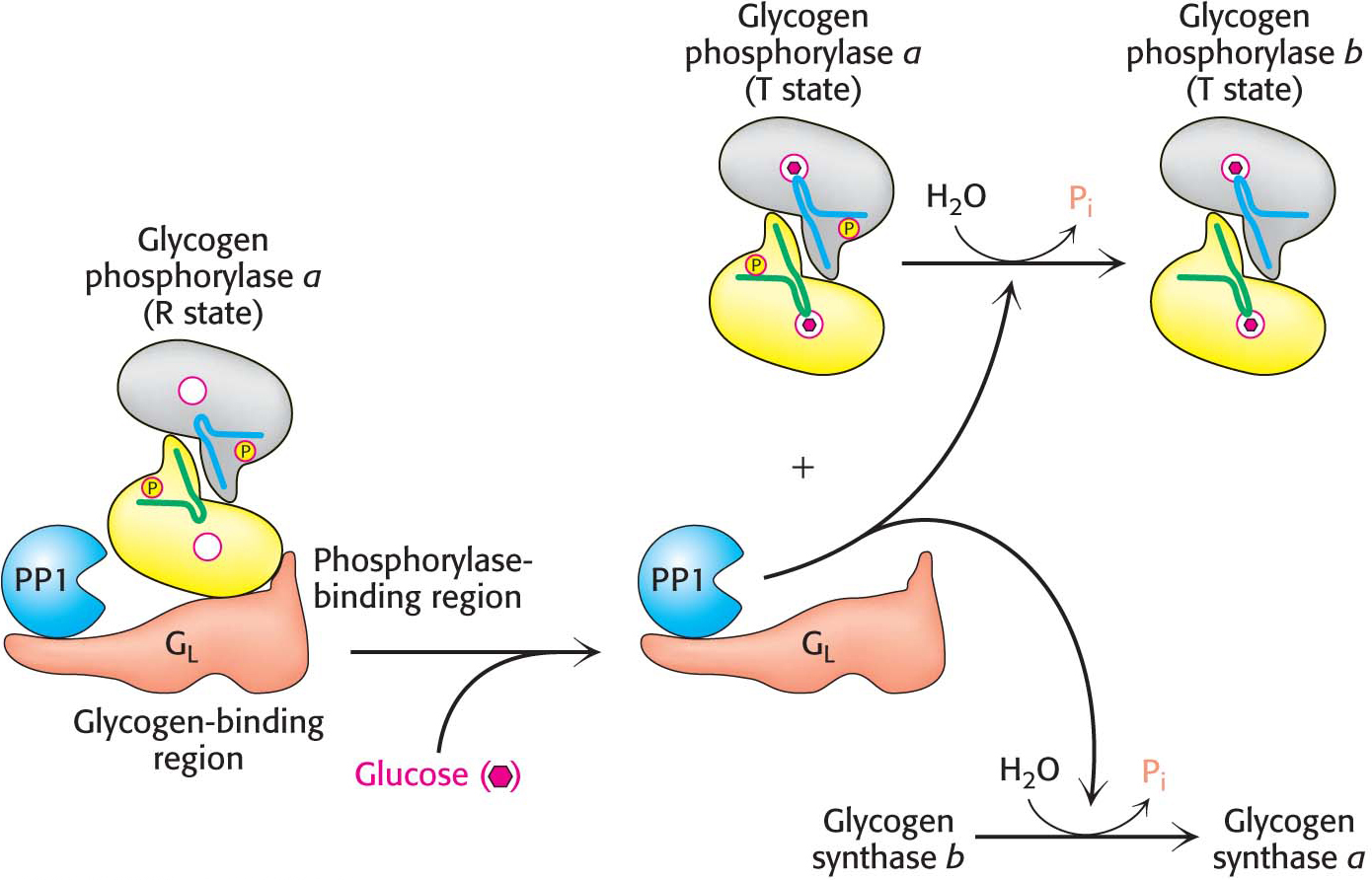
Figure 25.8: Glucose regulates liver-glycogen metabolism. In the liver, glucose binds to glycogen phosphorylase a and inhibits it, facilitating the formation of the t state of phosphorylase a. the t state of phosphorylase a does not bind protein phosphatase 1 (PP1), leading to the dissociation of PP1 from glycogen phosphorylase a and its subsequent activation. The free PP1 dephosphorylates glycogen phosphorylase a and glycogen synthase b, leading to the inactivation of glycogen breakdown and the activation of glycogen synthesis.
DID YOU KNOW?
Aretaeus, a cappadocian physician of the second century A.D., wrote: “the epithet diabetes has been assigned to the disorder, being something like passing of water by a siphon.” he perceptively characterized diabetes as “being a melting-down of the flesh and limbs into urine.” “Mellitus” comes from Latin, meaning “sweetened with honey.”
!clinic! CLINICAL INSIGHT: Diabetes Mellitus Results from Insulin Insufficiency and Glucagon Excess
Diabetes mellitus (usually referred to simply as diabetes) is a complex disease characterized by grossly abnormal fuel usage: glucose is overproduced by the liver and underutilized by other organs. The incidence of diabetes mellitus is about 5% of the world’s population. Indeed, diabetes is the most common serious metabolic disease in the world; it affects hundreds of millions of people. Type 1 diabetes, or insulin-dependent diabetes mellitus (IDDM), is caused by the autoimmune destruction of the insulin-secreting β cells in the pancreas and usually begins before age 20. Insulin dependency means that the affected person requires the administration of insulin to live. In contrast, most diabetics have a normal or even higher than normal concentration of insulin in their blood, but these diabetics are quite unresponsive to the hormone, a condition called insulin resistance. As discussed in Chapter 17, this form of the disease, known as type 2, or non-insulin-dependent diabetes mellitus (NIDDM), typically arises later in life than does the insulin-dependent form.
In type 1 diabetes, insulin production is insucient and consequently glucagon is present at higher-than-normal concentrations. Because insulin is deficient, the entry of glucose into adipose and muscle cells is impaired. The liver becomes stuck in a gluconeogenic and ketogenic, or fat-utilizing, state (Chapter 27). In essence, the diabetic person is in biochemical starvation mode despite a high concentration of blood glucose. The excessive amount of glucagon relative to that of insulin leads to a decrease in the concentration of fructose 2,6-bisphosphate (F-2,6-BP) in the liver. Hence, glycolysis is inhibited and gluconeogenesis is stimulated because of the reciprocal effects of F-2,6-BP on phosphofructokinase and fructose 1,6-bisphosphatase. The high glucagon-to-insulin ratio in diabetes also promotes glycogen breakdown. Hence, an excessive amount of glucose is produced by the liver and released into the blood. Glucose is excreted in the urine when its concentration in the blood exceeds the reabsorptive capacity of the renal tubules. Water accompanies the excreted glucose, and so an untreated diabetic in the acute phase of the disease is abnormally hungry and thirsty.
!clinic! CLINICAL INSIGHT: A Biochemical Understanding of Glycogen-Storage Diseases Is Possible
Edgar von Gierke described the first glycogen-storage disease in 1929. A patient with this disease has a huge abdomen caused by a massive enlargement of the liver. There is a pronounced hypoglycemia (low blood-glucose concentration) between meals. Furthermore, the blood-glucose concentration does not rise on the administration of epinephrine and glucagon. An infant with this glycogen-storage disease may have convulsions because of hypoglycemia.
The enzymatic defect in von Gierke disease was elucidated in 1952 by Carl and Gerty Cori, the husband and wife team who were awarded the Nobel Prize in 1947 for their work on glycogen metabolism. They found that glucose 6-phosphatase is missing from the liver of a patient with this disease. This finding was the first demonstration of an inherited deficiency of a liver enzyme. The liver glycogen is normal in structure but present in abnormally large amounts. The absence of glucose 6-phosphatase in the liver causes hypoglycemia because glucose cannot be formed from glucose 6-phosphate. This phosphorylated sugar does not leave the liver, because it cannot cross the plasma membrane. The presence of excess glucose 6-phosphate triggers an increase in glycolysis in the liver, leading to high concentrations of lactate and pyruvate in the blood. Patients who have von Gierke disease also have an increased dependence on fat metabolism. This disease can also be produced by a mutation in the gene that encodes the glucose 6-phosphate transporter. Recall that glucose 6-phosphate must be transported into the lumen of the endoplasmic reticulum to be hydrolyzed by phosphatase. Mutations in the other three essential proteins of this system can likewise lead to von Gierke disease.
Seven other glycogen-storage diseases have been characterized since von Gierke’s first characterization, and the biochemical reasons for the symptoms of these diseases have been elucidated (Table 25.1). In Pompe disease (type II), lysosomes become engorged with glycogen because they lack α-1,4-glucosidase, a hydrolytic enzyme confined to these organelles. The lysosomes sometimes burst, releasing glycogen into the cytoplasm (Figure 25.9). The Coris elucidated the biochemical defect in another glycogen-storage disease (type III) that now bears their name. In type III disease, the structure of liver and muscle glycogen is abnormal and the amount is markedly increased. Most striking, the outer branches of the glycogen are very short. Patients having this type of glycogen-storage disorder lack the debranching enzyme (α-1,6-glucosidase), and so only the outermost branches of glycogen can be effectively utilized. Thus, only a small fraction of this abnormal glycogen is functionally active as an accessible store of glucose.
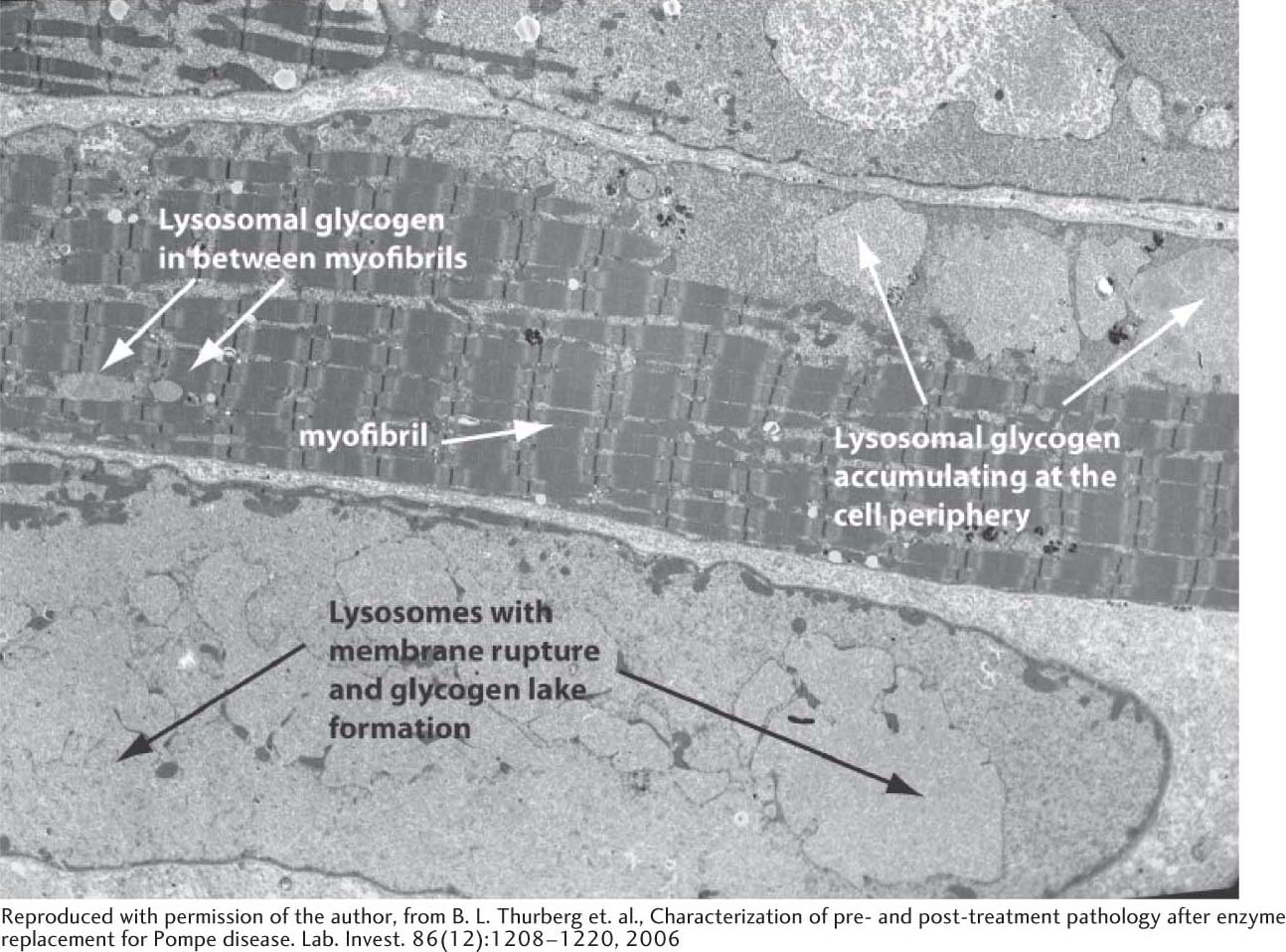
Figure 25.9: Electron micrograph of muscle cell from a patient with Pompe disease. Glycogen-engorged lysosomes are seen throughout the cell, including the myofibrils. As the disease progresses, lysosomes may rupture, releasing large amounts of glycogen into the cytoplasm. Such accumulations of cytoplasmic glycogen are called glycogen lakes.
A defect in glycogen metabolism confined to muscle is found in McArdle disease (type V). Muscle phosphorylase activity is absent, and a patient’s capacity to perform strenuous exercise is limited because of painful muscle cramps. The patient is otherwise normal and well developed. Thus, the effective utilization of muscle glycogen is not essential for life.
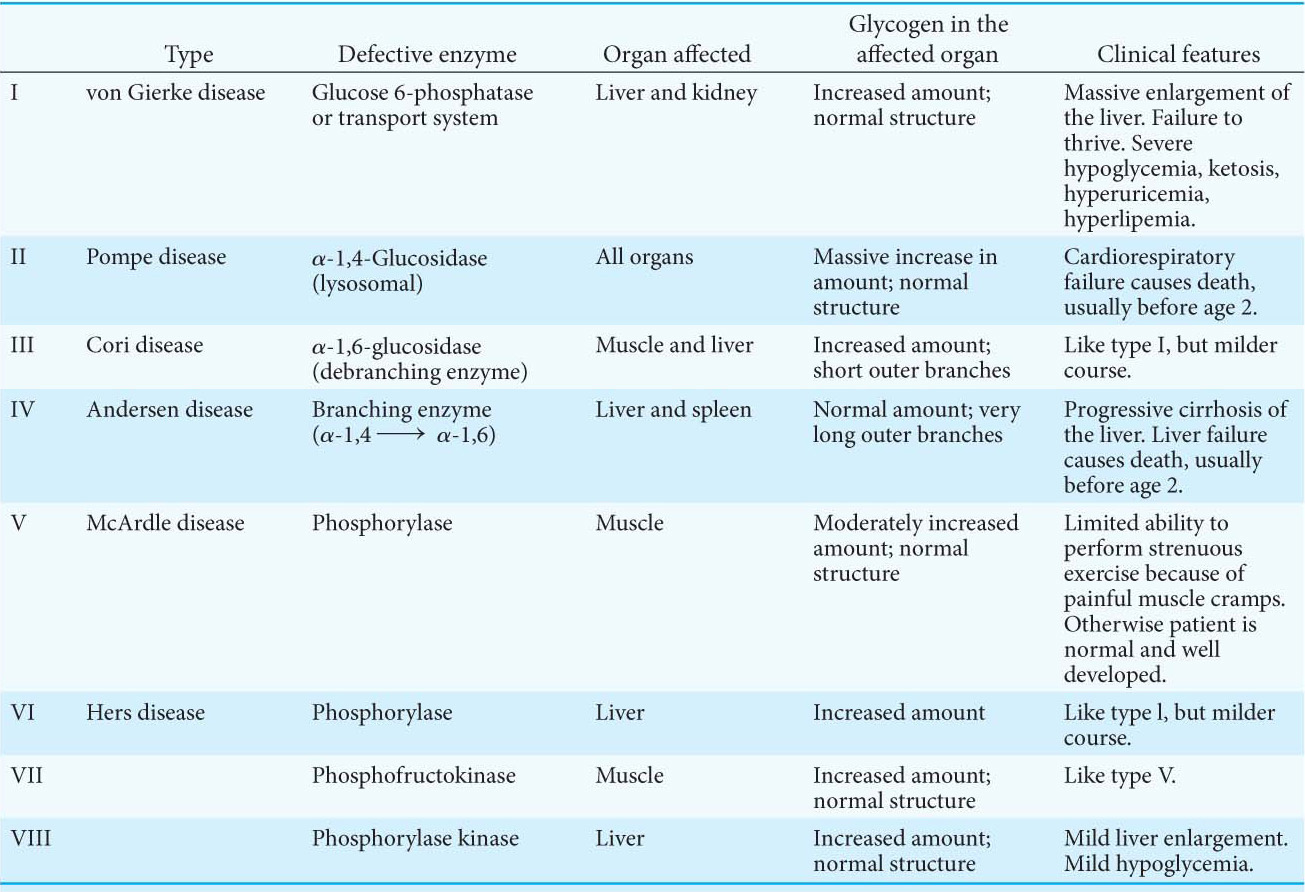
Table 25.1 Glycogen-storage diseases
Note: Types I through VII are inherited as autosomal recessives. Type VIII is sex linked.